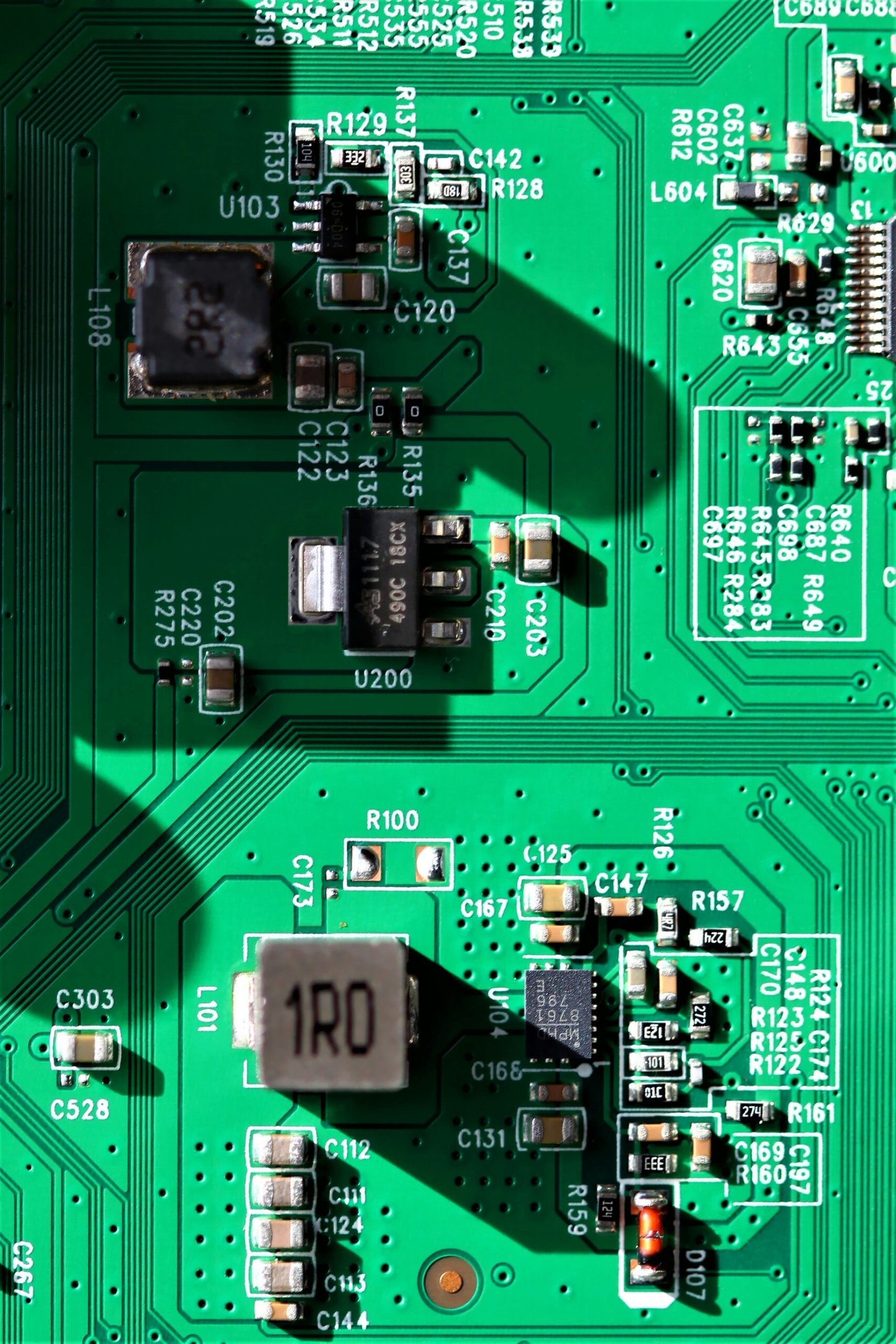
Introduction to Communication Signal Transfer
Communication signal transfer is a fundamental aspect of modern technology, serving as the backbone for various communication systems in use today. This process involves the transmission of information through electromagnetic signals, which include radio waves, microwaves, and light waves. The significance of effective communication signal transfer cannot be overstated, as it impacts everything from telecommunication networks to internet connectivity, enabling seamless interactions in a globalized world.
The role of electromagnetic signals in communication systems is crucial, as these signals are responsible for carrying data across vast distances. When we make a phone call, send an email, or stream a video, electromagnetic signals are employed to facilitate the transfer of information. However, the efficiency of this signal transfer is heavily dependent on the materials employed in the communication infrastructure. Traditional materials, while functional, often present limitations that can hinder signal quality and strength, leading to issues such as interference, attenuation, and bandwidth constraints.
Advancements in materials science have paved the way for engineered materials specifically designed to enhance signal transfer efficiency. These engineered materials, including crafted free electron materials, possess unique properties that allow for improved conductivity, lower attenuation, and the ability to operate across broader frequency ranges. As such, they hold the potential to revolutionize communication systems by ensuring higher-speed data transfer and more reliable connections.
In this blog post, we will explore how engineered free electron materials serve as a catalyst for improved communication signal transfer and discuss their applications in various technological domains. Through this exploration, we can gain insights into the future of communication technologies and the role of innovative material engineering in driving progress in this critical field.
Understanding Semiconductor Fundamentals
Semiconductors are essential materials used in modern electronics. At their core, the electronic behavior of semiconductors is governed by their energy bands, particularly the valence and conduction bands. The valence band is filled with electrons, while the conduction band is generally empty. The energy difference between these two bands is known as the band gap. This band gap is a critical property that determines the electrical conductivity of the material and its ability to act as a semiconductor.
When energy is supplied, such as through thermal energy or photon absorption, electrons can transition from the valence band to the conduction band. This movement facilitates current flow through the material, which is essential for the functionality of electronic devices. The size of the band gap significantly influences the semiconductor’s applications. For instance, materials with a small band gap are typically effective at room temperature, while those with larger band gaps are better suited for high-temperature applications.
Semiconductors can be categorized into two primary types: p-type and n-type. P-type semiconductors are created by adding acceptor impurities, which introduce “holes” in the valence band. These holes can accept electrons, resulting in positive charge carriers. Conversely, n-type semiconductors are formed by doping with donor impurities that introduce extra electrons into the conduction band, creating negative charge carriers. The interplay between these two types of semiconductors is fundamental in forming p-n junctions, the building blocks of many electronic and photonic devices.
In summary, understanding the foundational concepts of semiconductors, including the valence and conduction bands, the band gap, and the differences between p-type and n-type materials, contributes to grasping their pivotal role in revolutionizing communication through advanced signal transfer technologies.
Crafted Free Electron Materials: An Overview
Engineered free electron materials represent a significant advancement in the realm of materials science, particularly in communication technologies. These materials are designed to manipulate the behavior and properties of free electrons, which play a pivotal role in various electronic and optical applications. Crafted free electron materials are typically composed of a mixture of conductive elements, alloys, and nanostructures that enhance their efficacy in facilitating signal transfer.
The structure of these materials is essential for achieving desired electrical and optical properties. By controlling factors such as grain size, phase composition, and the distribution of nanoparticles, researchers are able to fine-tune the materials to optimize their performance. For instance, manipulating these parameters allows engineers to create materials that exhibit enhanced conductivity or specific plasmonic properties, which are crucial for effective signal transmission and reception in telecommunications.
One of the key properties of crafted free electron materials is their ability to support high carrier mobility, which enables fast electronic responses. These materials can be engineered to reduce scattering effects, thereby improving overall signal integrity. Furthermore, modifications in the chemical composition can yield materials that demonstrate specific optical behaviors, leading to novel applications in photonic devices.
Applications of crafted free electron materials are widespread, including use in terahertz systems, optoelectronics, and advanced communication networks. Their tailored designs allow for the development of devices that can operate efficiently at high frequencies, thereby revolutionizing communication methodologies. By leveraging engineered free electron materials, it is possible to exploit diverse phenomena, such as negative refraction and enhanced non-linear optical effects, ultimately improving the reliability and performance of modern communication systems.
The Importance of Band Structure in Frequency Response
The band structure of materials plays a pivotal role in determining their frequency response, significantly impacting the efficiency of signal transfer in communication systems. This allows for a better understanding of how different materials can be engineered to enhance communication capabilities, especially as demands for increased data throughput continue to grow. The band gap, which is the energy difference between the valence band and the conduction band in a material, is crucial in defining its electronic properties. A smaller band gap generally allows for higher electron mobility, enabling faster response times across a range of electromagnetic frequencies.
When the band structure is optimized, materials can efficiently transmit signals across various frequency ranges. This phenomenon is particularly important in the context of engineered free electron materials, which are specifically designed to manipulate their band structures for enhanced signal transfer. Materials with tailored band gaps can be employed to improve their performance in specific frequency ranges, which is essential for applications such as telecommunications and high-speed data transfer.
Moreover, the relationship between band structure and electron mobility is essential for understanding how materials respond to varying frequencies. High electron mobility materials can support rapid signal transmission, reducing latency and improving overall performance. In contrast, materials with limited electron mobility may hinder signal propagation, leading to increased losses and reduced communication efficiency. Thus, material performance is inextricably linked to its band structure and the underlying electron dynamics.
This correlation underscores the importance of conducting thorough investigations into the band structures of potential materials. As research progresses, developing innovative materials with optimized electronic properties will progressively advance the field of communication systems. By meticulously engineering band gaps and enhancing electron mobility, next-generation materials can be realized, paving the way for superior signal transfer capabilities necessary in modern technological landscapes.
P-Type and N-Type Semiconductor Materials: A Comparative Study
P-type and N-type semiconductor materials serve as the foundation for modern electronic devices, each with distinct properties and functions pivotal in signal transfer and communication technologies. Both types of semiconductors are created through a process known as doping, which involves adding specific impurities to pure materials to alter their electrical conductivity. P-type semiconductors are formed by introducing elements that have fewer valence electrons than the semiconductor substrate, typically silicon. This process creates “holes” or positive charge carriers, allowing for the flow of electricity. Common dopants for P-type materials include boron and gallium.
In contrast, N-type semiconductors are developed by adding elements with more valence electrons, which result in excess electrons that act as negative charge carriers. Phosphorus and arsenic are typical examples of dopants used in N-type materials. The charge carriers play a critical role in how these materials function within electronic circuits; P-type materials facilitate the movement of holes, while N-type materials enable the flow of electrons. As such, they often work in tandem, resulting in comprehensive electronic components such as diodes and transistors.
Applications for P-type and N-type semiconductor materials are widespread in communication devices. For instance, in mobile phones and computers, transistors built from these materials manage signal amplification and switching, essential for efficient data transmission. Similarly, light-emitting diodes (LEDs) often rely on both semiconductor types to produce light through electroluminescence. The performance and integration of P-type and N-type semiconductors are critical for improving signal transfer capabilities, leading to faster and more reliable communication in today’s digital world.
The Emergence of 2D Materials in Communication Technologies
In recent years, two-dimensional (2D) materials have garnered significant attention due to their unique properties that make them particularly advantageous for communication technologies. These materials, consisting of a single layer of atoms, exhibit remarkable characteristics such as exceptional electrical conductivity, mechanical strength, and flexibility. The most prominent example of a 2D material is graphene, a monolayer of carbon atoms arranged in a hexagonal lattice. Other noteworthy 2D materials include transition metal dichalcogenides (TMDs) and black phosphorus, each offering distinctive traits that contribute to advancements in signal transfer.
The thinness of 2D materials enables them to enhance electromagnetic interactions in weak fields, which can lead to improved signal transmission efficiency. Unlike traditional bulk materials that may hinder the performance of electronic devices, 2D materials allow for better integration within circuits. This integration is crucial, especially as the demand for faster and more reliable communication escalates. For instance, the unique band structure of TMDs facilitates the development of high-performance photodetectors and transistors, which are essential components in modern communication systems.
Furthermore, engineered 2D materials can be tailored to exhibit specific properties, thereby optimizing them for various applications. The ability to manipulate these properties through chemical doping or structural modifications opens new avenues in technology, allowing for the creation of devices that operate more efficiently in diverse conditions. Additionally, the lightweight and flexible nature of these materials paves the way for innovative applications in wearable technology and flexible electronics, thus broadening their scope in communication applications.
As research into 2D materials continues to advance, their potential to transform communication technologies becomes increasingly clear. The ongoing exploration and engineering of these ultrathin materials promise to address current challenges in signal transfer, ultimately leading to more efficient, robust, and adaptable communication systems.
Shaping Electromagnetic Interaction with New Material Lattices
Recent advancements in engineered materials, particularly two-dimensional (2D) material lattices, have opened new avenues for enhancing electromagnetic interactions. These sensitive materials can significantly influence signal transfer, especially in weak electromagnetic fields where traditional materials might struggle to maintain performance. By leveraging unique structural properties at the atomic level, the engineered 2D lattices exhibit extraordinary capabilities in manipulating electromagnetic waves, which directly correlates to improved communication protocols.
The mechanism behind the modulation of electromagnetic interactions by these novel lattices is rooted in their ability to interact with incident electromagnetic fields in a compliant manner. When electromagnetic waves encounter 2D material lattices, the lattice structures can induce localized fields, enabling stronger signal retention and amplification. This property is paramount for applications requiring precise signal control in complex environments, thereby advancing communication systems’ capabilities.
Moreover, the tunability of these 2D materials allows for real-time adjustments in their interaction characteristics. For instance, by altering their configurations—be it through external strain or changing temperature—the electromagnetic response can be fine-tuned to optimize signal transfer characteristics actively. This adaptability provides engineers with a powerful tool for enhancing the performance of communication networks through the efficient management of signals that are typically difficult to handle.
As we look toward the future of communication technologies, the implementation of these advanced engineered materials promises significant improvements. Enhanced material properties in 2D lattices could lead to more efficient data transmission, reduced energy consumption, and higher resilience against interference. In turn, this would enable the development of more robust and reliable wireless communication systems, ultimately revolutionizing how we communicate in an increasingly interconnected world.
Challenges and Opportunities in Material Science for Communication
The integration of engineered free electron materials into communication technologies presents a unique set of challenges and opportunities. One of the most significant challenges is scalability. As the demand for faster and more efficient communication systems increases, it becomes imperative to develop materials that can be produced at a large scale without compromising their performance. Current manufacturing processes for engineered free electron materials often involve complex techniques that can limit output and raise costs, thus hindering widespread adoption in the telecommunications sector.
Additionally, the manufacturing complexity of these materials raises concerns about consistency and reliability. Variations in the production process can lead to defects or inconsistencies in material properties, which directly affects the signal transfer efficiency and overall performance of communication devices. The industry requires innovative methodologies that simplify the production of free electron materials while ensuring their stability and effectiveness in real-world applications.
Material stability is another pressing concern. Communication technologies need materials that can withstand diverse environmental conditions and exhibit longevity in high-performance scenarios. Engineered free electron materials may suffer from degradation over time, which could diminish their effectiveness in various communication settings. Thus, research and development initiatives must prioritize the formulation of materials that resiliently maintain their properties over extended periods.
Despite these challenges, there are significant opportunities for innovation. Advances in nanotechnology and material science can pave the way for creating engineered free electron materials that not only meet the operational demands of modern communication systems but also enhance their capabilities. Collaborative efforts between academia and industry can lead to breakthrough discoveries that will revolutionize the way signals are transferred, thereby reinforcing the potential of engineered materials in transforming communication technologies for the future.
Conclusion and Future Directions
In the rapidly evolving landscape of communication technologies, engineered free electron materials have emerged as pivotal components facilitating enhanced signal transfer. The significance of these materials lies not only in their inherent properties but also in their potential to shape the future of communication systems. As we have explored, their unique characteristics enable the manipulation of signals at unprecedented speeds and efficiencies, positioning them as integral elements in the quest for reliable and effective communication.
Looking forward, the field of materials science is poised for remarkable advancements, particularly regarding quantum communications and next-generation networks. As researchers delve deeper into the capabilities of engineered materials, we can anticipate innovative solutions to current communication challenges. This encompasses developing materials that can operate effectively at quantum levels, thereby enabling secure and rapid data transmission. The integration of such materials into next-generation network architectures will likely support the evolving demands of global connectivity.
Moreover, the pursuit of new engineered free electron materials is expected to drive significant research directions. This may include exploring novel compositions and structures to enhance signal fidelity and reduce latency in communication systems. Active collaboration between material scientists, engineers, and communication experts will be essential to translate theoretical discoveries into practical applications. The commitment to innovation within this field will undoubtedly lead to breakthroughs that transform how we establish connections and share information.
In conclusion, as we navigate through an era defined by digital transformation, the role of engineered free electron materials cannot be overstated. Their contributions to signal transfer are foundational for the future of communication, emphasizing the necessity for ongoing research and exploration in this domain. The potential that lies within these materials invites optimism and curiosity about the communication technologies of tomorrow.